Second-harmonic generation and manipulation in lithium niobate slab waveguides by grating metasurfaces
Download: 715次
1. INTRODUCTION
Since optical harmonic generation was first observed by Franken et al. [1] in a single-crystal quartz in 1961, nonlinear optics has achieved enormous developments, which have a wide range of significant applications in sensors [2], wavelength converters [35" target="_self" style="display: inline;">–
Despite LN’s excellent linear and nonlinear optical properties, its intrinsic inert chemical properties and physical hardness have prevented the development of LN nanophotonics. Fortunately, inspired by the silicon-on-insulator (SOI) technology in the semiconductor industry, which has promoted the evolution of compact photonic integrated devices and circuits [13], -on-insulator (LNOI) substrates have been commercially available in recent years. The LN thin film is located on top of the low-index buffer layer, fabricated via ion slicing and crystal bonding [14]. Due to the high refractive index contrast between and the substrate, it is efficient for confining the light into subwavelength scale and offers an opportunity for dense integrated devices. Since then, there has been encouraging progress in fabricating diverse thin-film LN optical devices [15
In this work, we experimentally demonstrate SHG as well as beam shaping in LN slab waveguides on a monolithic z-cut LNOI based on grating metasurfaces. Instead of using previous poling technology or precisely engineering the dispersion properties and geometric features of the waveguide, we directly fulfill the phase-matching condition via a simple grating metasurface that can implement the nonlinear process from the free-space fundamental wave to the guided second-harmonic (SH) wave. Moreover, holographic designs are further introduced in the metasurface on the slab waveguide, which enables flexible nonlinear beam shaping. We experimentally demonstrated multifocusing and Airy beam SHG, promising multichannel nonlinear conversion and generation for optical interconnections.
2. DESIGN AND SIMULATIONS
In our study, the LNOI platform is employed with a single crystal z-cut LN thin-film layer with a thickness of 300 nm, underneath which is a 1.8 μm thick substrate. Owing to the high index contrast and the subwavelength thickness of the LN film, the waveguide modes are well confined into a small scale. The film is considered a slab waveguide in our scheme. As illustrated in Fig.
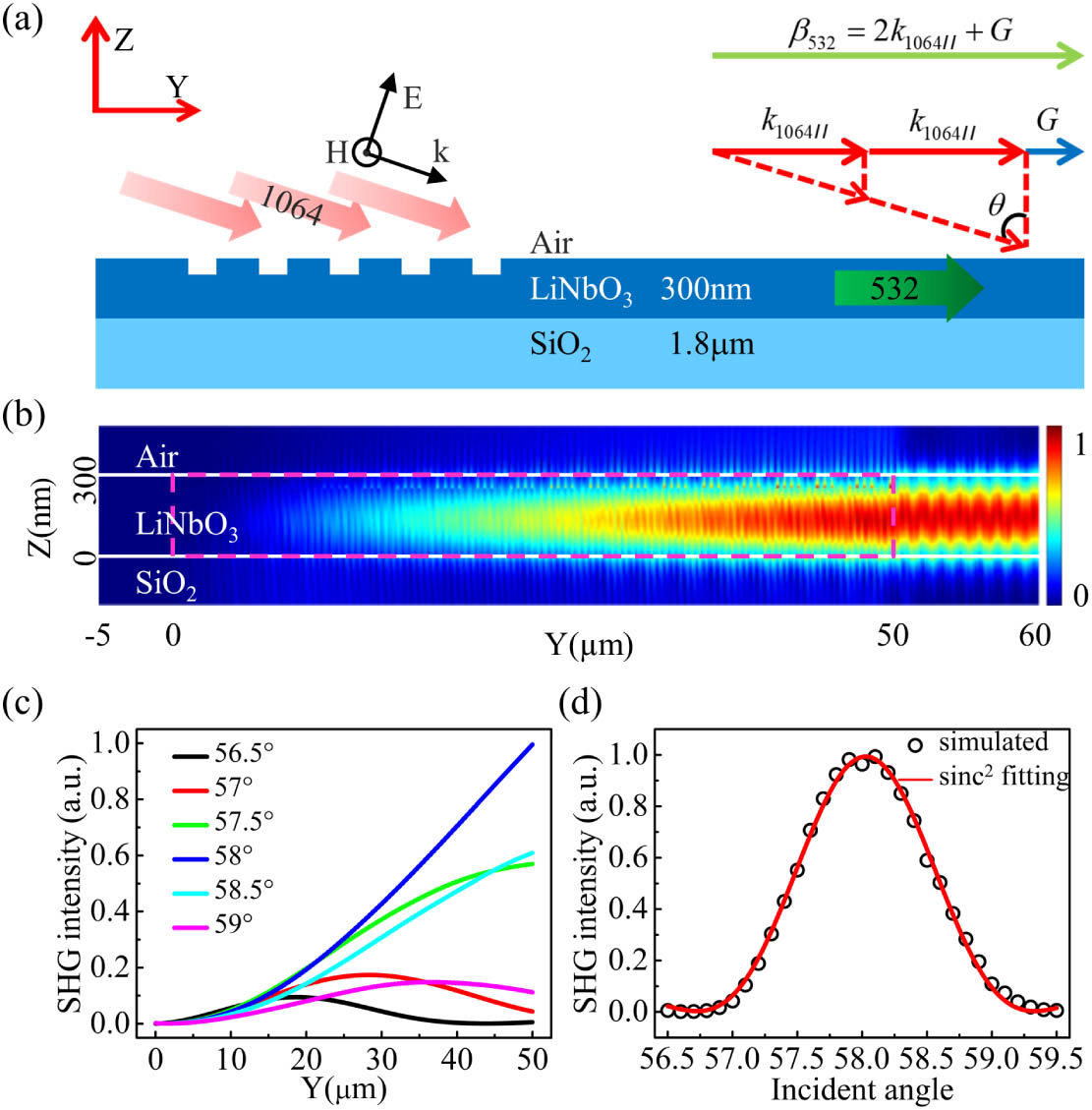
Fig. 1. Schematic illustration of the phase-matching process and the simulations. (a) Theoretical principle of the proposed SHG in the LN slab waveguide. (b) Simulated evolution of the electric field in the waveguide at the SH frequency, indicating an efficient SHG process. The grating distributed region is emphasized with the pink box. (c) Simulated SHG intensity versus propagation length with different incident angles . (d) Simulated -dependence of the SHG on the incident angle.
In principle, there are several parameters that can be optimized to access the phase-matching condition. Here we mainly want to provide a proof-of-concept demonstration and will not focus too much on parameter optimization. For simplicity, we fix several parameters. In detail, the grating period , the duty cycle , the etching depth , the length of the coupling region , the width of the coupling region , and thus the TM pump incident angle can be adjusted for the best phase-matching condition. Figure
3. FABRICATION AND MEASUREMENTS
In experiments, we fabricated the slab waveguides patterned with grating metasurfaces by a focused ion beam (FIB, dual-beam FEI Helios 600i) with acceleration voltage of 30 kV and beam current of 40 pA. Due to the poor electrical conductivity of LN, a 50 nm thick Ag layer was first deposited on the bare thin-film top surface via ion beam sputtering, and it was removed in dilute after the FIB etching. Figure

Fig. 2. Experimental demonstration of the SHG in the slab waveguide. (a) SEM images of the fabricated samples with Ag cladding. (b) Schematic illustration of the measurement setup. (c) Measured SHG power at the output port of samples with different lengths of the coupling regions, in comparison with the simulations. (d) Quadratic power dependence between the pump and the SH signal in the experiments.
A femtosecond laser with (Fianium, pulse width of , repetition rate of 40 MHz) is employed for illumination with an incident angle of . A collection of an objective lens and a group of filters is placed before the electron multiplying charge coupled device (EMCCD, Andor, iXon Ultra 888) to detect the SHG signals as they are output from the waveguide’s diced facet as in the optical setting shown in Fig.
In addition, to show the in-plane manipulation of the generated SH wave, we fabricated a 90°-turn reflection Bragg grating (period with a 45° angle) to the left side of the 100 μm sample with a distance of 200 μm. Below this reflector, an out-of-plane grating coupler (period ) was made with a distance of 200 μm in the direction. In this case, the 1064 nm light is incident with an opposite inclined angle (from right to left) onto the grating metasurfaces, and thus the SHG wave will propagate to the left direction. The generated 532 nm SH wave will be reflected by the Bragg reflector and propagate to direction as in the coupled out signal shown in the inset figure [bottom left in Fig.
4. MANIPULATION OF THE SHG GUIDED WAVE
More importantly, our method offers a powerful tool in manipulating the wavefront of the SH signals inside the waveguide without extra devices. The two-dimensional hologram is encoded in the grating metasurfaces by the following equation [36,37]: This equation describes a binary modulated grating based on holographic technology, where is the etching depth of the grating, and is the period in the direction of propagation , which satisfies the phase-matching condition. where contains the information of the amplitude, and is the desired initial phase encoded in the transverse direction in order to control the guided mode to follow a special trajectory in propagation.
According to the hologram design, we have fabricated another three samples by FIB with different SHG beam shaping functions (area of the coupling region of ). In detail, we obtain the desired phase and amplitude by holography. We consider the target wavefront as an object wave, and it interferes with the incident SHG plane wave. For multifocal lens designs, the holographic field of the sources in the axis of gratings can be calculated by summarizing all the radiations from the focus as [38,39] where is the distance between the focus and the source position in the axis, and is the amplitude of the focus. For one focal lens () and dual focal lens (with distance of 50 μm) designs, we obtain the phase function and keep the amplitude function ] constant, which means the amplitude is uniform. In order to realize the Airy beam inside the waveguide, we use the following expression for the electric field distribution [40]: Here Ai is the Airy function and is a transverse scaling factor, here chosen to be 2.048 μm for demonstration. The complex result of Eq. (
Figures

Fig. 3. Manipulation of SHG guided wave. Zoomed SEM images of structures for (a) an ordinary plane wave, (b) a focal lens, (c) a dual-focus lens, and (d) an Airy beam generator in the waveguide. (e)–(h) Measured light spot intensity profile coupling out from the facet of waveguides for four different beam manipulations. (i)–(l) Corresponding vertical cross sections of the light spots (black solid curves), in comparison with the simulation results (red dashed curves).
5. DISCUSSIONS AND CONCLUSION
Honestly speaking, the total efficiency of our approach is quite low as compared with previous works. However, it is reasonable since our approach merges several processes together, and the efficiency is not purely determined by the nonlinear conversion process within the waveguide. In our case, the fundamental wave is traveling in the free space with a certain efficiency to be coupled into a guided mode with a simultaneous nonlinear conversion, which indeed decreases the total efficiency remarkably. A possible solution to increase the coupling efficiency is to add a reflector below the slab-waveguide layer to capture more reflected pumping light, or even to construct a cavity in the vertical dimension to further enhance the interaction of incident light with the nanostructures.
In summary, we have demonstrated a new scheme to achieve nonlinear wavelength conversion and beam shaping in slab waveguides in the LNOI platform. With flexible grating metasurface designs, we convert the fundamental wave from free space into a manipulated guided SH wave, instead of the previous two or more steps, making the system simpler and more compact. Furthermore, the holographic grating metasurfaces enable us to manipulate the generated SH signals with one more degree of freedom, and we can control their wavefront in the waveguide as desired. Thus, we also demonstrated several functions in nonlinear beam shaping, like focusing, dual focusing, and the Airy beam generation, which offer opportunities for flexible on-chip multichannel routing. Our approach is promising to enable new exciting possibilities in integrated nonlinear nanophotonic designs and devices.
6 Acknowledgment
Acknowledgment. T. Li is grateful for the support of Dengfeng Project B of Nanjing University.
[4] J. P. Meyn, C. Laue, R. Knappe, R. Wallenstein, M. M. Fejer. Fabrication of periodically poled lithium tantalate for UV generation with diode lasers. Appl. Phys. B, 2001, 73: 111-114.
[7] T. Udem, R. Holzwarth, T. W. Hänsch. Optical frequency metrology. Nature, 2002, 416: 233-237.
[12]
[13] B. Jalali, S. Fathpour. Silicon photonics. J. Lightwave Technol., 2006, 24: 4600-4615.
[30] R. Luo, Y. He, H. Liang, M. Li, Q. Lin. Semi-nonlinear nanophotonic waveguides for highly efficient second-harmonic generation. Laser Photon. Rev., 2019, 13: 1800288.
[36] W. H. Lee. Binary computer-generated holograms. Appl. Opt., 1979, 18: 3661-3669.
Article Outline
Bin Fang, Hanmeng Li, Shining Zhu, Tao Li. Second-harmonic generation and manipulation in lithium niobate slab waveguides by grating metasurfaces[J]. Photonics Research, 2020, 8(8): 08001296.