Dynamic relaxation process of a 3D super crystal structure in a Cu:KTN crystal
Download: 947次
In the past half century, potassium tantalate-niobate (KTN) crystals have attracted a lot of attention in the research and application field because of their exceptional electro-optic (EO) properties and wide transparent wavelength range[1
Among all the mentioned phenomena, the 3D super crystal has become a hotspot. That phenomenon appears in a KTN sample with a built-in one-dimensional (1D) compositional oscillating seed[22,23]. In this kind of KTN, as the paraelectric-ferroelectric phase transition takes place, simultaneously that built-in 1D order is transferred to the whole volume with the same spatial scale. The formed 3D structure can cause an optical diffraction phenomenon similar to typical X-ray diffraction since the scale of this structure and the wavelength of visible light are numerically close, just as the relationship of a crystal lattice and X-ray[24].
Theory of paraelectric-ferroelectric relaxation phase transition is not certainly defined[19], and an important method to clarify it is to measure the relaxation time of PNRs/polarized clusters. With the measured relaxation time, the relaxation process can be described by a sum of relaxation functions. A 3D super crystal is essentially dynamic relaxation, which can be illustrated by some kinds of relaxation functions with a main characteristic relaxation time[19,2527" target="_self" style="display: inline;">–
The Cu-doped KTN,
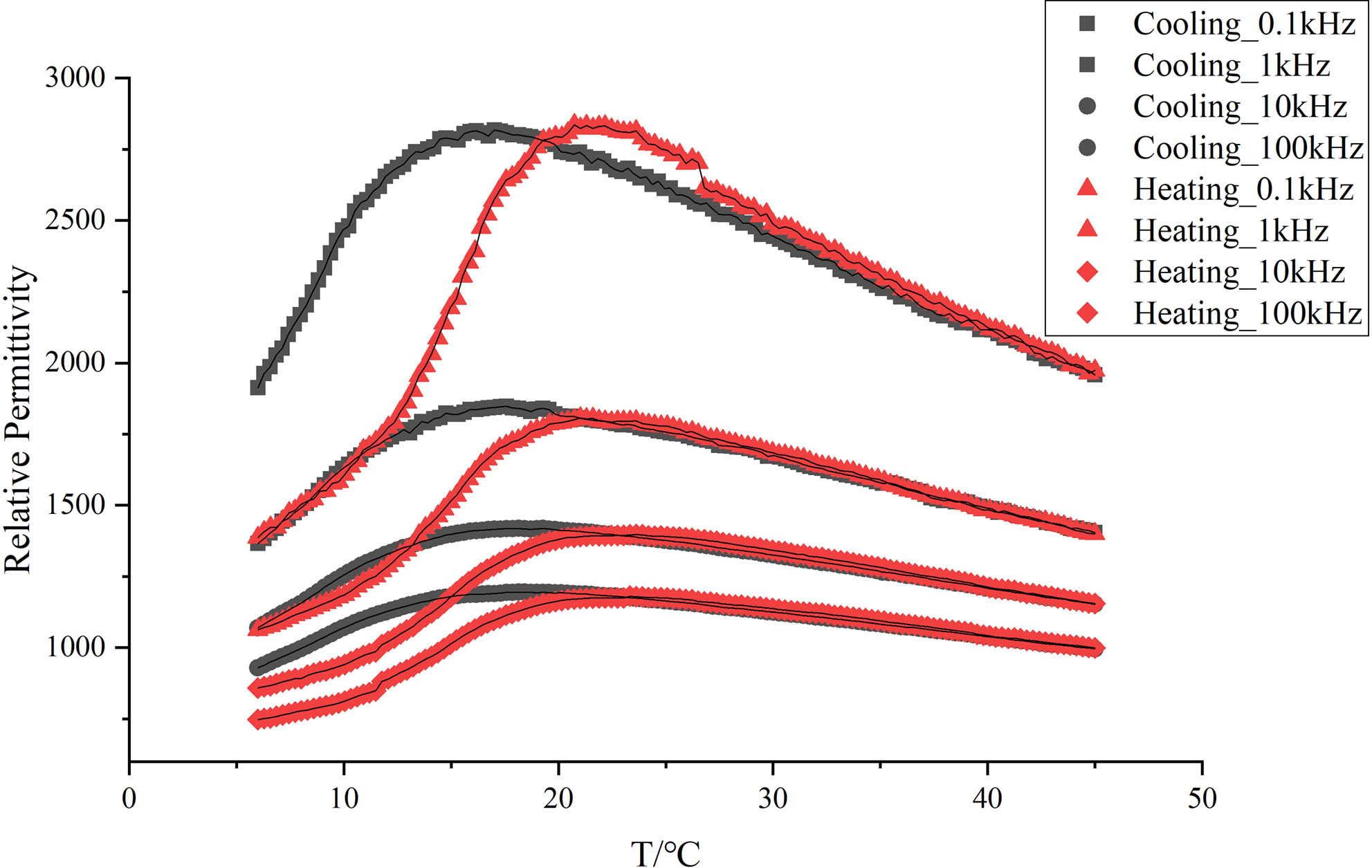
Fig. 1. Variation of relative permittivity against temperature and measurement frequency. Measurement is accomplished by a Tonghui 2830 LCR (inductance, capacitance, resistance) meter with 1Vpp sinusoidal signals at different frequencies. Thermal hysteresis loops are shown because of the crystal’s relaxor ferroelectric. As shown, data at decreasing temperatures are smoother, and as frequency increases, the whole intensity decreases without a change of curve shapes.
The whole process works in the following way[29,30]. Initially, a laser beam goes through a polarizer and enters the crystal sample, and then suffers a diffraction effect caused by the 3D super crystal[26]. Then diffraction light with a specific diffraction angle travels through the second polarizer (which has a vertical polarization angle with respect to the first one) and then is collected by a photomultiplier tube (PMT). The signal is transferred to a computer for further processing and analysis, as shown in Fig.

Fig. 2. Sketch of the DLS experiment setup. A light beam with a 532 nm wavelength generated by a laser is first polarized and then shot into the crystal sample. After suffering strong diffraction in the sample, the light beam changes its direction. The pinholes and filter behind the sample make sure a light beam with a special direction can get through. Two polarizers with a V-H (vertical and horizontal) setup can pick up the light signal that has been influenced by the super crystal structure. To increase the capturing efficiency of the light signal, a lens is localized in front of the PMT.
As microstructures of the 3D super crystal in the sample are undergoing relaxation dynamics[16,17], the intensity of scattering light gained by the PMT will fluctuate. So, in the time domain, information about the time scale of the structure evolution can be obtained in the fluctuating signal that the PMT outputs.
The second-order autocorrelation function can be generated from the scattering light intensity as[30]
A laser beam at 532 nm (consistent with the response interval of the PMT) is generated by a Coherent Verdi G-Series laser. The temperature of the sample is controlled by a semiconductor chilling plate with Arroyo Instruments 5305 TEC Source, which has an accuracy of 0.01°C. A Thorlabs PMT1001/M with a lossless response frequency up to 80 MHz collects light intensity data and outputs pulse signals to a Keysight DSOX6004A oscilloscope for staging.
The whole system is separated from the external environment by optical barriers. Relaxation time data corresponding to the formation process of the super crystal begin to be recorded once the temperature reaches 11.5°C. For the disruption process of the super crystal, the data begin to be recorded once the external field is applied. For characteristic relaxation time measurement, the data collection begins 10 min after the temperature reaches 11.5°C to avoid influences from the generation process. After each measurement, the sample has been heated to 50°C and kept for 15 min to eliminate the polarization history and fatigue, and then cooled to 11.5°C for the next measurement. The change rates for heating and cooling are both 3°C/min.
An important procedure of data analysis is to fit the autocorrelation function (ACF). As we all know, Debye relaxation describes the dielectric relaxation response of ideal dipoles[21]. Here we introduce the Kohlrausch–Williams–Watts (KWW) function (a variant of the Debye relaxation function) to further delineate the relaxation process[33,34]. In the time domain, the formula is
The PMT outputs ∼10 ns pulses and the time-resolved counts of pulses represent the average light intensity in each little time zone. Though we have got the light intensity variation with time, it is so tiny compared with the full intensity of the signal that the ACF has to be applied to gain it. Actually, the ACF calculated directly is relatively rough, and we do a re-average operation on it to acquire clear consequence. The whole calculation task is accomplished by MATLAB scripts. Raw data with scripts are available for proper purpose.
As introduced in method descriptions, there exists an intrinsic 1D grating, also called component concentration modulation along the z direction inside the as-grown crystal[16,18]. Kept at 50°C, the sample is highly transparent for its cubic phase, and its spontaneous polarization and polarization history are basically eliminated[35]. Now most incident laser beams directly propagate through the sample with the native vector direction, while a few are diffracted by the intrinsic 1D grating structure and form

Fig. 3. (a)–(c) Diagram of the 3D dynamic super crystal and the X-ray-diffraction-like phenomenon. (a) A diagram of a dynamic 3D super crystal structure full-filling the whole column. Due to 1.52 μm period that is very similar to the wavelength of the incident light beam (532 nm), the diffraction pattern is convenient to observe and analyze. (b) Strong diffraction caused by the dynamic structure. The built-in 1D order (periodical refractive index variety) is also shown. (c) Picture of diffraction pattern captured behind the sample. Spots pointed out by blue circles correspond to the -order Bragg diffraction caused by the intrinsic 1D grating, and the spot pointed out by red circle corresponds to the diffraction phenomena caused by domain walls. The latter is utilized in the DLS experiment. (d)–(f) Microscopic mechanism during dynamic structure formation. (d) PNRs with orientation vertical to the interface of different layers form. (e) PNRs with orientation vertical to the interface have strong spontaneous polarization, and those with orientation horizontal to the interface have little during dynamic relaxation process. (f) The final dynamic structure. Red lines represent 90° domain walls.
The microcosmic mechanism of the 3D super crystal formation process is described here. When the sample first reaches 11.5°C (
The characteristic relaxation time of this kind of super crystal with respect to the KWW function is 7.75 s and 32.93 s, which means there are two kinds of relaxation models with different orders of magnitude of time. Here, the stretching parameter of the KWW function used in the fitting process is

Fig. 4. Characteristic relaxation time calculated by fitting the ACF of two kinds of experiment data, equals 7.75 s and 32.93 s, respectively.
Characteristic relaxation time data measurement begins after the scattering light intensity is stable. Two kinds of relaxation time with different orders of magnitude of time are obtained with different data collection times: relaxation time of 7.75 s is with a data collection time of 1 min; that of 32.93 s is with a data collection time of 10 min and above. There is no valid result for shorter data collection times. We also make some measurement during the super crystal formation and disruption process. Gained relaxation times are calculated to be 49.59 s and 22.05 s for the formation and disruption process, respectively. These results share a same order of magnitude of time with the characteristic relaxation time.
As we can see, the obtained relaxation time corresponding to the intrinsic relaxation process of a 3D super crystal is longer than that of polarization clusters and PNRs, though the 3D super crystal structure is essentially formed by them. The order of magnitude of the time difference between the 3D super crystal dynamics and the original relaxation ferroelectric dynamics results from the strong coupling and interaction of the polarization clusters and PNRs. At the proper critical temperature (
Since relaxation ferroelectric materials have many incredible properties compared with general ferroelectric materials, the relaxation mechanism has become a foundational physical theory. However, though many researchers have spent lots of efforts, a perfect theorem is still absent. Our work shows that with strong coupling, interaction of microcomponents, and stickiness of a critical glassy mosaic phase disorder background, a 3D super crystal exhibits a much longer relaxation time feature compared with the inside microcomponents. Therefore, a 3D super crystal as a novel spontaneous phenomenon not only is foreseeable to be used in functional diffraction devices but also gives us a brand-new vision of the ferroelectric relaxation mechanism.
[1]
[2]
[3]
[4]
[5]
[6]
[7]
[8]
[9]
[10]
[11]
[12]
[13]
[14]
[15]
[16]
[18]
[19]
[20]
[21]
[22]
[23]
[24]
[25]
[26]
[27]
[28]
[29]
[30]
[32]
[33]
[34]
[35]
Quanxin Yang, Xin Zhang, Hongliang Liu, Xuping Wang, Yingying Ren, Shan He, Xiaojin Li, Pengfei Wu. Dynamic relaxation process of a 3D super crystal structure in a Cu:KTN crystal[J]. Chinese Optics Letters, 2020, 18(2): 021901.