Omnidirectional iridescence via cylindrically-polarized femtosecond laser processing
1 Introduction
A distinct type of coloration found in nature comes as a result of the analysis of the incident light to individual wavelengths realized by sophisticated periodic or pseudoperiodic constructions present within the body of natural species1. As a result, the so-called structural colors originate from the fundamental optical processes of diffraction, interference or scattering and exhibit attractive features, including iridescence, angle- and polarization-dependent response. Most important structural coloration is permanent and durable contrary to the pigment coloration, which is sensitive to environmental factors such as heat, UV radiation, and interaction with chemicals. As a consequence, structural colors have attracted significant research interest in the field of biomimetics2.
Owing to their unique optical properties, bioinspired diffractive surfaces with multiple periodic structures are commonly used as diffracting elements for a plethora of scientific and industrial applications, such as color and holographic displays, telecommunication3, cosmetics4, anti-counterfeiting4-6, spectroscopy and color analysis systems, solar cells7, and biomedical devices. Structural coloration on technical surfaces is typically achieved by patterning the surface to form gratings exhibiting periodicities on the order of the visible wavelengths. Nowadays, several techniques have been employed to texture materials in the micro- and submicrometer range including electron-beam lithography and etching process8, or ablation with focused ion beam9. Among the mask-free fabrication methods, laser-based direct patterning techniques are proved to be promising for structural coloration, as they are simple and single-step processes10-12. In particular, surface processing with pulsed lasers has been used for the manufacturing of submicron periodic structures that appear within the laser beam limits after irradiation13-17. In particular, the fabrication of LSFL in the form of parallel fringes with great spatial accuracy and homogeneity are possible upon irradiation with ultrashort laser pulses, due to the limited collateral damage and thermal effects. The formation mechanism of these periodic structures has been investigated by many researchers18-21. LSFL are thoroughly characterized by their depth, periodicity, and orientation. Both periodicity and depth are dependent on the fundamental characteristics of the laser irradiation such as wavelength, intensity, and scanning speed17. However, their orientation is absolutely dependent on the polarization state of the laser beam22. Specifically, for metallic surfaces, LSFL align perpendicularly to the incident net force of the E-field components for a typical linearly polarized pulse23-26. Vorobyev and Guo27, 28 have demonstrated the possibility of realizing well-defined structural color via a precise control of periodicity and orientation of LSFL, upon changing various irradiation parameters. Following this work, there are numerous reports on the fabrication of structural colors via laser based fabrication approaches10-12, 29-32, 33.
Nevertheless, none of the methods reported to date, including the laser-based ones, is capable to produce multidirectional spatial frequency patterns, in a single step. As a consequence, the produced iridescence strongly depends on the viewing angle, thus it exists only for specific angles of incidence and is absent upon tilting the diffraction element34, 35. Besides this, it has been both experimentally and theoretically reported, that diffraction cannot occur when the plane of light incidence is parallel to grating orientation23, 36. Efforts have been made in order to overcome the above restrictions with the generation of LSFL in variable orthogonal directions by utilizing the polarization dependence of the ripple orientation. In particular, a grid can be fabricated where each laser scanning direction employs different polarization state such as p and s polarization respectively36, 37. Therefore, the surface can produce diffraction in two different planes of incidence. Also, it has recently been reported that irradiation with circular polarization pulses can form triangular periodic surface structures that could potentially significantly increase the range of incident light-illumination angles38. Despite these efforts, omnidirectional iridescence has not yet been realized via a single-step irradiation process.
In this paper we present, an effective, simple and single-step technique for the fabrication of diffractive surfaces, exhibiting iridescence with great efficiency for any angle of incidence. This is realized via large-area processing of steel with radially polarized cylindrical vector (CV) fs laser beams25, 39, leading to the formation of LSFL, which are spatially oriented in multiple directions. It is observed that for practically every possible incident angle of illumination, the plane direction of light is always perpendicular to locally formed LSFL, hence reflective diffraction can take place. Τhe ability of the obtained structures to act as diffraction gratings was systematically studied and compared against the diffraction originating on the conventional ones fabricated using linearly polarized laser pulses.
2 Materials and methods
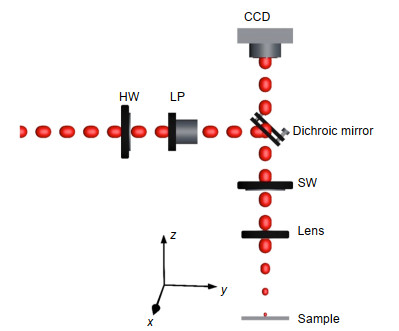
Fig. 1. The experimental setup used for surface structuring.The laser fluence was tuned via the use of a λ /2 waveplate (HW) and a linear polarizer (LP). Laser source emits a Gaussian linearly polarized beam, which is transformed to a CV radially polarized beam by means of an s-waveplate (SW). Large areas were produced via translation of the sample in the x -y -z directions.
where R is the Gaussian beam radius, while for CV beams, R is the outer and r the inner radius. In the case of area scanning at constant velocity v, repetition rate f and distance between successive scanning lines (δ), Neff-area can be calculated from:
The morphology of the periodic surface structures has been characterized by scanning electron microscopy (SEM, JEOL JSM-7500F). In order to determine the LSFL periodicity and directionality, SEM images were analyzed via two-dimensional fast Fourier transform (2D-FFT), using the Gwyddion software.
To investigate the influence of the angle of an incident white light on the structural color formation, a diffraction characterization setup was built, as shown in
3 Results and discussion
The generation of LSFL with the best possible uniformity depends strongly on the irradiation parameters. For this purpose, a comprehensive parametric study was conducted via line scanning, to investigate the effect of F and Neff on the topographical features induced. The parametric study led to the creation of optimized, well-organized and uniform LSFL structures under specific irradiation conditions for both polarization states.

Fig. 2. LSFL periodicity dependence on the laser fluence (a), and the effective number of pulses (b), for linearly (squares) and radially (circles) polarized fs beams. Top-view SEM images of areas produced upon irradiation using linearly (c, d) and radially (e, f) polarized fs beams.The images shown in (c) and (e) correspond to non-uniform areas, whereas those in (d) and (f) to areas obtained using optimized irradiation conditions. The red arrows depict the electric field polarization state. The 2D-FFT patterns corresponding to each area are shown.
It is observed that the LSFL produced via CV beams exhibit lower periodicities, which is in accordance to our former findings and interpretation25, 43. Furthermore, in both S1 and S2 cases, the periodicity is weakly affected by the laser fluence.
Large areas of 5 mm × 5 mm were also fabricated via scanning the fs beam onto the sample surface using the optimized conditions found during the line scanning experiments.

Fig. 3. Top-view SEM images of areas produced upon irradiation using linearly (a–c) and radially (d–f) polarized fs beams.The images shown in (b, c) and (e, f) are higher magnifications of the images (a, d) respectively. The areas in (a–c) were fabricated at F =0.45 J/cm2 and Neff-area=36, δ =32 μm, and v =2 mm/s, while those in (d–f) were obtained at F =0.49 J/cm2, N eff-area=30, δ =34 μm, and v =2 mm/s. The red arrows depict the electric field polarization state. The 2D-FFT patterns corresponding to each area are shown in the insets.
The average period calculated by the FFT pattern for the linear-polarized case equals to 870±80 nm. On the other hand, areal scanning with CV beams gives rise to more complex LSFL structures exhibiting multi-directional orientation (
Following irradiation, all the processed surfaces exhibited vivid coloration, which is expected, considering that the calculated LSFL periodicities are close to the visible wavelengths44, 45. It is also striking that the S2 samples are iridescent at practically any viewing angle, contrary to the S1 ones.
where λ is the diffracted wavelength, Λ corresponds to the LSFL period, and m is the order of diffraction, in our case m=±1. The angle φ denotes the white light incidence, while ω corresponds to the sample rotation within the sample plane. Assuming that θ is the sample tilt angle, the grazing angle equals to φ+θ. The ability of the surface to act as a diffraction grating was tested for eight different angles ω, starting from 0° to 360° with a step of 45°. For each angle ω, the grazing angle was changed accordingly and the corresponding coloration was captured by a camera, placed along the z-axis. The angle β was also recorded for each measurement.

Fig. 4. (a ) Experimental setup and geometry used for the evaluation of surface diffraction properties. (b ) Typical intensity plot of the white light spectrum. (c ) Schematic illustration of the structural color monitoring system. (d ) The respective coordination parameters.

Fig. 5. Schematic illustration of the structural colors observed the S1 (a) and the S2 (b) sample series respectively; 2D-FFT patterns corresponding to the S1 (c) and the S2 (e) sample series respectively. The corresponding intensity plots of the 2D-FFT patterns in four different directions (denoted as '1' to '4') are depicted in (d) and (f) respectively.Inset in (f) displays the periodicity values of LSFL structures for a series of four cross-sections taken in the Fourier image of the S2 surface.
As mentioned above, the diffraction properties and respective structural colors are quite different for the S2 series of surfaces fabricated by a radially polarized CV beam. This is due to the formation of multi-directional LSFL structures, giving rise to reflective diffraction, regardless of the rotational angle ω. As a result, the surface is iridescent for practically any viewing angle, although the vividness and sharpness of the diffracted colors are slightly different depending on the angle ω. Specifically, for the angles ω = 0°, 90°, 180°, and 270°, the diffracted colors appeared to be most vivid and exhibit the maximum rendering capacity, compared to the angles ω
= 45°, 135°, 225°, and 315°. This effect is also indicated by the respective FFT pattern as shown in

Fig. 6. (a ) Diffracted colors of S1 surfaces obtained upon irradiation with linearly polarized fs beam. (b ) Diffracted colors of S2 surfaces obtained upon irradiation with radially polarized fs beam. Each color is characterized by the coordinates (ω , φ +θ , β ), as defined by the optical system used for their monitoring.
4 Conclusions
We have presented a novel technique for the fabrication of large areas of multi-directional laser-induced periodic surface structures exhibiting omnidirectional diffraction properties. It is based on LSFL formed upon exploiting the cylindrical symmetry of CV fs field. The diffraction and structural coloration properties are by far different than those exhibited by LSFL areas fabricated via linearly polarized Gaussian beams. Our results show that it is possible to efficiently tune the diffraction and structural coloration properties via surface processing strategies employing femtosecond CV beams. We envisage that this work will pave the way for the realization of customized photonic components and devices.
5 Acknowledgements:
This work was supported by MouldTex project-H2020-EU.2.1.5.1 (GrantAgreement No. 768705).
6 Competing interests
The authors declare no competing financial interests.
[2] C S S R Kumar. Biomimetic and Bioinspired Nanomaterials. Wiley, Weinheim, 2010.
[4] E G Loewen, E Popov. Diffraction Gratings and Applications. M. Dekker, New York, 1997.
[6] N P Mahalik. Micromanufacturing and nanotechnology. Berlin, Heidelberg: Springer Berlin Heidelberg, 2006.
[44] Hecht E.
[45] Palmer C.
Article Outline
Nikolaos Livakas, Evangelos Skoulas, Emmanuel Stratakis. Omnidirectional iridescence via cylindrically-polarized femtosecond laser processing[J]. Opto-Electronic Advances, 2020, 3(5): 05190035.