All-optical PtSe2 silicon photonic modulator with ultra-high stability
Download: 827次
1. INTRODUCTION
All-optical modulation has received extensive attention since it avoids the conversion of electrical signals to optical signals [1]. Although electrically driven modulators using such as charge carrier injecting effect [2], thermo-optic effect [3], and Pockels effect [4] have achieved great success on silicon and lithium niobate platforms, all-optical modulation still plays an irreplaceable role due to its simple structural design and easy manufacturing process. Meanwhile, novel two-dimensional (2D) materials emerging in recent years, such as graphene [5], black phosphorus (BP) [6], and transition metal dichalcogenides (TMDs) [7], provide more opportunities to implement all-optical signal processing, with respect to their distinct merits, such as intrinsic lattice matching with silicon, complementary metal oxide semiconductor integrability, as well as excellent electrical, optical, and thermal properties [8,9]. Li et al. demonstrate all-optical intensity modulation in a graphene-clad microfiber by using Pauli blocking in graphene; an ultra-fast decay time of 2.2 ps and a modulation depth of 38% are achieved [5]. Thermo-optic effect [10] is also used to achieve all-optical phase modulation in the microfiber assisted by graphene [11], BP [6], MXene [12], or tungsten disulfide () [7], but the response time is of the order of milliseconds. The integrated photonic platform offers higher compactness and potential for manufacturing large-scale photonic integrated circuits [13,14]. All-optical modulation based on an integrated platform has been intensively studied using photo-excited free-carrier concentrations [15], electromagnetic induced transparency [16], reverse saturable absorption [17], or photothermal effect (assisted by metal–insulator–metal absorbers) [1820" target="_self" style="display: inline;">–
As a group of representative 2D materials, TMDs are composed of two chalcogen (X) atomic layers and a transition metal (M) atomic layer sandwiched between them [25]. Compared with the zero bandgap and weak optical absorption (2.3%) of monolayer graphene [26], TMDs have bandgaps that change with the number of layers and strong resonant absorption at specific wavelengths [27]. In addition, TMDs can be prepared by the chemical vapor deposition (CVD) method and other bottom-up growth techniques, which reveal the advantages of excellent uniformity, controllable number of layers, strong reliability, high repeatability, and large area preparation [28,29].
Platinum selenide (), a new type of layered TMD, has been intensively studied due to its special properties since it was first epitaxially grown by direct selenization of Pt on a substrate [30]. Its crystal structure can be regarded as being composed of hexagonal closely arranged Se atoms with Pt atoms occupying octahedral sites in alternate Se layers [25]. Different from other TMDs with large bandgaps (such as monolayer ) [31,32], monolayer has a smaller indirect bandgap of 1.2 eV, while the bilayer directly reduces to 0.21 eV [25,33]. If the thickness continues to increase, it will eventually become a Type II Dirac semimetal without a bandgap [30,3436" target="_self" style="display: inline;">–
In this work, we propose and experimentally demonstrate an all-optical modulator with a 2D -on-silicon structure. The material is grown with the CVD method and then transferred onto a silicon microring resonator (MRR). By using a 980 nm laser to irradiate the surface of the device from free space, we successfully demonstrate all-optical modulation based on the photothermal conversion of PtSe2. A tuning efficiency of is achieved. The dynamic 10%–90% rise/decay time is 304/284 μs, and the 3 dB bandwidth is measured to be 2.4 kHz. We also perform a comparative experiment on a pure silicon device and confirm that the photothermal conversion efficiency almost entirely comes from the 2D . Due to the outstanding air stability of , the tuning efficiency of the fabricated device only decreased by 7.5% after being exposed to the air for 3 months, which exhibits the long-term stability of this all-optical modulator. Results show that the -based device is a potential option to be deployed in all-optical signal processing, showing great advantages in low cost and easy fabrication.
2. DEVICE FABRICATION AND PRINCIPLE
The optical microscopy image of the as-prepared film is illustrated in Fig.
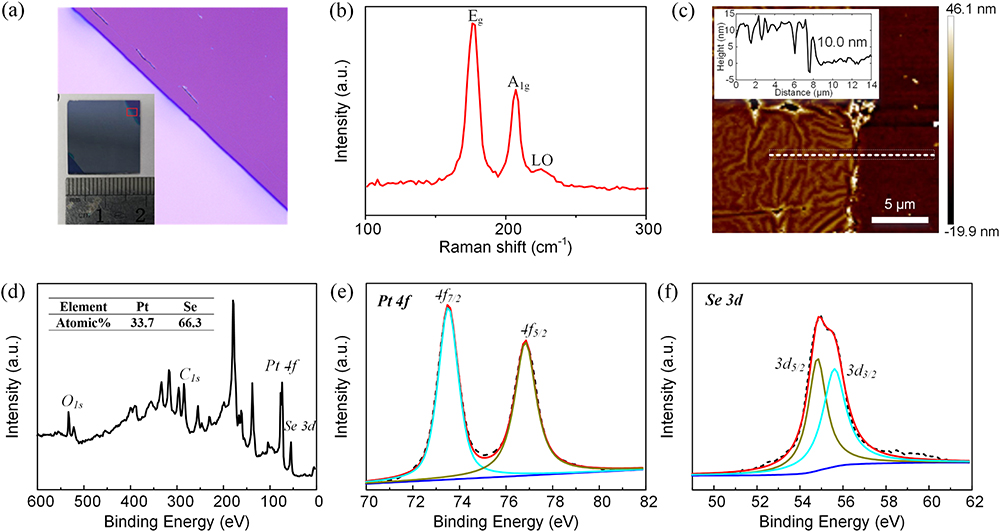
Fig. 1. (a) Optical microscopy image of the CVD grown film. (b) Raman spectrum of film. (c) AFM image of the transferred and the typical height profile along the white dashed line. XPS spectra of (d) the film, (e) the Pt 4f region, and (f) the Se 3d region.
The microscope images of the all-optical MRR modulator are displayed in Figs.

Fig. 2. (a) Microscope image of the all-optical modulator where film fully covers the whole device. (b) A zoom-in on the part of the MRR shows uniformly transferred thin film. (c) Cross section of the -on-silicon structure. (d) Transmission spectra of the MRR without (brown) and with (blue) the film (normalized respectively). (e) All-optical modulation mechanism and experimental setup of the -based modulator. TLS, tunable laser source; ASE, amplified spontaneous emission; PC, polarization controller; SMF, single-mode fiber; OSA, optical spectrum analyzer; PD, photodetector; OSCP, oscilloscope.
Figure
The resonance wavelength of the MRR is given by Eq. (
3. EXPERIMENTAL SETUP
The experimental setup of the proposed all-optical modulator is schematically shown in Fig.
4. RESULTS AND DISCUSSION
4.1 A. Experimental Results
The static pump results are shown in Fig.

Fig. 3. (a) Static spectral response for different pump powers. (b) Measured resonance shift versus pump power and the corresponding linear fitting curves, for pristine device (red) and the device after 3-month air exposure (blue).
The pump power from 0 to 210.9 mW (measured by a hand-held power meter, FPM-300 Power Meter, EXFO) is applied to measure the static response of the device when a 980 nm laser source works in the CW mode. The results in Fig.
To characterize the dynamic response of the device, the 980 nm pump laser operates in the 100 Hz SW mode with a power of 210 mW [Fig.

Fig. 4. (a) Pump light with 100 Hz frequency and (b) the corresponding modulated signal light. (c) Several signal waveforms at different frequencies. (d) V pp versus modulation frequency.
4.2 B. Discussions
In order to evaluate the true photothermal efficiency of the , the photothermal conversion efficiency of the modulator is calculated by combining the thermo-optic and thermal expansion theory of silicon with Eq. (

Fig. 5. Infrared thermograms of the modulator with pump powers of (a) 50 mW and (b) 500 mW. (c) Surface temperature change of the modulator versus pump power.

Fig. 6. (a) Optical microscopy image of the pure silicon MZI. (b) Static spectral response for different pump powers. (c) Measured resonance shift versus pump power and the corresponding fitting curve.
Here a comparison of the reported all-optical modulators based on the photothermal effect of 2D materials is summarized in Table
Table 1. Comparison of Reported All-Optical Modulators Based on the Photothermal Effect of 2D Materials
|
Therefore, it is possible to achieve more efficient pumping by using a lensed fiber tip to reduce the size of the spot [20]. The silicon-based thermo-optic modulator has achieved a response time of 0.6 μs [3], which means accurate material etching and specific optimized air gaps that can help the heat distribution to be concentrated on the waveguide rather than spread far away [20], which may further improve the modulation efficiency and response speed of the device. In addition, the demanded spectral shift and the corresponding pump power can be reduced by optimizing the factor of the device. The optical losses of the device are mainly attributed to the contamination during the process of material transferring and the absorption caused by the material covering the grating couplers and other non-pumping parts, which can be eliminated by patterning definition [54].
5. CONCLUSION
In conclusion, we propose and demonstrate an all-optical modulator with a -on-silicon structure. By utilizing the photothermal effect of , we successfully convert the information from pump light to probe light. The device achieves a modulation bandwidth of 2.4 kHz with a 10%–90% rise/decay time of 304/284 μs, and the tuning efficiency is . Thanks to the long-term stability of in the air, the tuning efficiency of the device keeps almost unchanged after 3 months. This work brings 2D to the field of silicon-based photothermal modulation devices for the first time and shows its great potential for the all-optical information processing field, such as optical routing, switching, and logic gate and sensing, with low cost and simple manufacturing process.
6 Acknowledgment
Acknowledgment. The authors also acknowledge the support from Instrumental Analysis Center of Shenzhen University (Xili Campus).
[10] K. Wu, Y. Wang, C. Qiu, J. Chen. Thermo-optic all-optical devices based on two-dimensional materials. Photon. Res., 2018, 6: C22-C28.
[21] Z. Shi, L. Gan, T.-H. Xiao, H.-L. Guo, Z.-Y. Li. All-optical modulation of a graphene-cladded silicon photonic crystal cavity. ACS Photonics, 2015, 2: 1513-1518.
[24] H. Wang, N. Yang, L. Chang, C. Zhou, S. Li, M. Deng, Z. Li, Q. Liu, C. Zhang, Z. Li, Y. Wang. CMOS-compatible all-optical modulator based on the saturable absorption of graphene. Photon. Res., 2020, 8: 468-474.
[45] K. Zhang, M. Feng, Y. Ren, F. Liu, X. Chen, J. Yang, X. Yan, F. Song, J. Tian.
Article Outline
Kangkang Wei, Delong Li, Zhitao Lin, Zhao Cheng, Yuhan Yao, Jia Guo, Yunzheng Wang, Yupeng Zhang, Jianji Dong, Han Zhang, Xinliang Zhang. All-optical PtSe2 silicon photonic modulator with ultra-high stability[J]. Photonics Research, 2020, 8(7): 07001189.