Surface-illuminated photon-trapping high-speed Ge-on-Si photodiodes with improved efficiency up to 1700 nm
1. INTRODUCTION
Timely development of cost-effective and power-efficient optical interconnects is necessary to meet the high demand of data transfer in the era of the Internet of Things (IoT) that is expected to connect billions of sensors with different functionalities [1,2]. Datacenters are envisioned to scale up to meet the high demand of connectivity. Intra- and inter-datacenter communications require optical links for reach gap (500 m–2 km), long-reach (
In addition, applications such as quantum communications [8], eye-safe lidar systems [9], and photonic biosensors [10], require detectors operated at the near-infrared, particularly at 1310 or 1550 nm, taking advantage of the low-loss windows of optical fibers and low scattering of light at those wavelengths in the atmosphere and tissue, respectively.
Commercially available optical receivers often contain photodiodes (PDs) based on III–V materials such as InGaAs/InP [11,12]. However, these materials are not compatible with CMOS technology and would incur additional costs for wafer bonding, packaging, yield, thermal management, etc. [13,14]. On the other hand, monolithic integration of PDs with all electronics on a single chip, fully hermetic and without ceramic multichip carriers for the receiver end, can reduce the cost dramatically. Ge/Si devices provide a possible solution for these kinds of high-speed applications, as BiCMOS SiGe technology has already been proved in CMOS foundries [15]. In addition, low field transport is much faster in Ge than in Si, and high field transport is similar between both materials. Thus, using Ge for the i-layer in a pin PD is highly advantageous in improving speed [16].
Although bulk Ge has a very broad absorption spectrum, the direct bandgap of Ge is only 0.8 eV, which results in weak absorption at and beyond 1500 nm. However, the absorption coefficient of Ge can be greatly enhanced beyond 1500 nm by introducing tensile strain in the epitaxial Ge film on an Si substrate due to the different coefficients of thermal expansion (CTEs) [17,18]. Recent developments of Ge epitaxy on Si substrate [19], including
Among the surface-illuminated photodiodes, resonant cavity enhanced (RCE) Ge PDs can exhibit both high external quantum efficiency (EQE) and high speed [27], but they are also wavelength specific and sensitive to temperature, which makes them unfavorable for optical receivers, particularly in WDM applications. Waveguide-type Ge PDs have long optical length and a short carrier collection route, so they can realize high EQE and high speed at the same time. However, due to the narrow width of the waveguide, they need tight fiber alignment, which can add additional cost [14]. On the other hand, a conventional surface-illuminated pin PD is limited by the EQE and bandwidth product as there is often a trade-off: smaller absorption thickness leads to a fast but low efficiency device, while larger absorption thickness results in an efficient but slow device. As light travels vertically into the PDs, the EQE is determined by the thickness of the absorption region (intrinsic Ge) where photons get absorbed [shown in Fig.
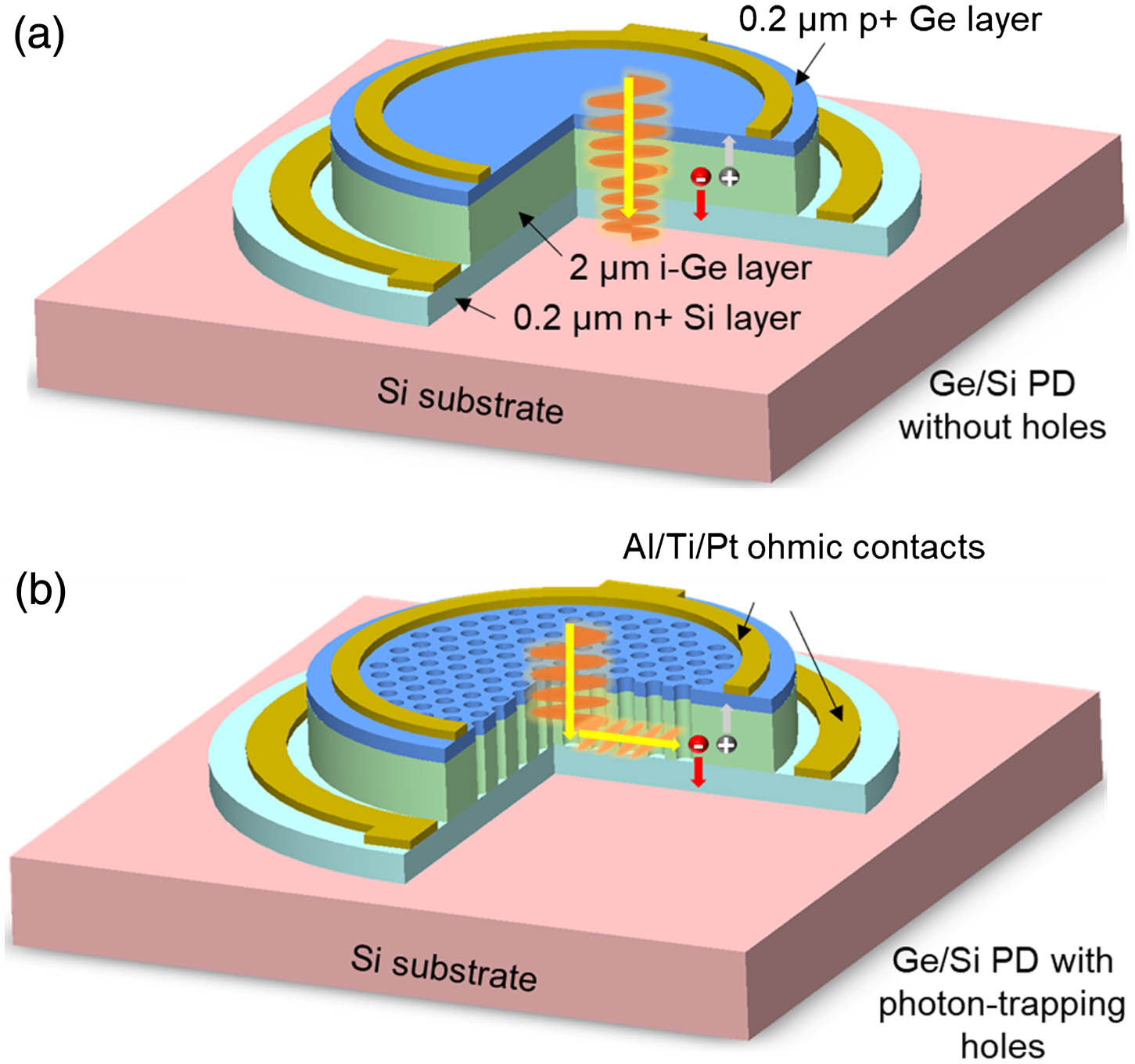
Fig. 1. Schematics of Ge/Si PD active layers (a) without holes and (b) with photon-trapping holes. The Ge-on-Si PD is composed of 0.2 μm p+Ge (blue), 2 μm i-Ge (green), and 0.2 μm n+Si (silver) layers. The yellow rings are the Al/Ti/Pt ohmic contacts. The red circle with the “−” sign and the dark circle with the “+” sign represent photon-generated electron and hole, respectively.
2. LIGHT GUIDING WITH HOLE ARRAYS
Indirect bandgap semiconductors such as Si and Ge require a relatively thick material along the propagation direction of the incident light for effective absorption of wavelengths that have energies close to the bandgap edge of the semiconductor. The required thickness is usually of the order of the inverse value of the absorption coefficient (

Fig. 2. (a) Schematic diagram that shows the enhanced optical path in a slab with hole arrays by light guiding near-perpendicular to the incoming light compared to an optical path in a bulk semiconductor in the direction of incident light. The effective absorption coefficient of a slab with hole arrays becomes much larger than the bulk absorption coefficient of the semiconductor due to the enhanced optical path. A thin slab with hole arrays can be used in a fast photodiode without losing efficiency, whereas a thick bulk semiconductor causes the photodiode to operate at slow speed in order to work efficiently. Typical field distributions around microholes at (b) , (c) , (d) , and (e) , showing light propagation in the lateral direction inside the material. FDTD simulations were performed for hexagonally packed tapered holes with diameter/period of 1150/1750 nm at 1550 nm wavelength for a 1.1 μm depletion layer (to be consistent with fabricated PDs demonstrated in Section 4.B ). The top and bottom rows show cross sections and top views of the electric field. The border of a hole with a taper at the top is represented by white lines. (f) Simulated electric field intensity of light at 1550 nm wavelength, propagating from air into a Ge-on-Si slab without hole arrays at . There is no sign of lateral light propagation, and light is propagating in the direction of incidence throughout the film with less intensity due to a higher surface reflection.
On the other hand, a semiconductor slab with periodic hole arrays, as shown in Fig.
As incident light illuminates the structure, guided modes are formed due to periodic arrays of holes [30]. Such modes have propagation constants in both the vertical and lateral directions. These guided modes trap light in the high refractive index Ge material between the air and silicon and enhance the reflection of light at the Si/Ge interface. In addition to light trapping, the hole arrays also reduce the surface reflection by reducing the refractive index difference between air and the Ge surface. Periodic holes with tapered sidewall angles can introduce a graded refractive index profile, which allows light to enter the structure smoothly.
In this study, we used the finite-difference time-domain (FDTD) method to simulate light propagation in our device structures. A periodic boundary condition was used in the directions parallel to the PD’s surface, while the boundary in the direction of incident light (perpendicular to the PD’s surface) was assumed to be a perfectly matched layer (PML). A set of Lorentz models was used to simulate wavelength-dependent absorption [31]. The model based on the Green’s function formalism [32] was utilized in FDTD simulations for strained Ge film on Si substrate.
Light propagation in a structure with periodic hole arrays can be different from that in a slab without holes. Constructive and destructive interference of light in the incident direction is expected as light travels through a thin slab. However, a structure with periodic hole arrays interacts with light in a way that a lateral component of light propagation occurs in addition to the vertical component of propagation in the incidence direction. Figures
Figure

Fig. 3. (a) Calculated absorption of Ge-on-Si PDs with (red line) and without (black line) tapered holes (diameter/period: 1150/1750 nm) arranged in a hexagonal lattice for a wavelength range of 1200–1800 nm. A 0.2% strain in the Ge layer is considered during calculations. The simulated structures have a 2 μm i-layer as shown in Fig. 1 . While Ge-on-Si PDs without holes theoretically absorb until 1550 nm, PDs with holes potentially keep high absorption of light up to 1700 nm. The green and blue lines represent absorption of PDs with and without holes, respectively, in the case of relaxed Ge layer as a comparison. 0.2% strain does not cause a significant difference for PDs with holes, whereas there is some improvement in absorption of PDs without holes toward longer wavelengths when a 0.2% strain is introduced to the Ge layer. (b) The calculated transmission and reflection (inset) for PDs with (red lines) and without (black lines) holes. The green and blue lines represent the case of a relaxed Ge layer as a comparison.
Figure
3. DEVICE STRUCTURE AND FABRICATION
The pin layers of Ge photodiodes were epitaxially grown on an Si substrate. A 2 μm thick intrinsic Ge layer is sandwiched between a highly phosphorus doped (
All the fabrication processes for the Ge photodiodes are CMOS compatible and were conducted in a class 100 cleanroom. The as-grown Ge-on-Si wafer was first cleaned in PRS3000 solution to remove any organic contaminant. Then, 0.9 μm i-line resist was uniformly spin-coated onto the Ge-on-Si wafer. GCA 8500 i-line stepper was used as the exposure tool to pattern the i-line resist. Similar to the reactive ion etch (RIE) technique performed on silicon described in Refs. [28,29], funnel-shaped microholes were created on the Ge surface, as Fig.

Fig. 4. (a) Top view scanning electron microscope (SEM) image of funnel-shaped holes on the Ge surface. The holes are 1.1 μm in diameter and 1.2 μm in period, and in a hexagonal lattice. (b) Cross-sectional SEM image of the funneled holes showing sidewall angle of around 65°. The Ge and Si interface is also clearly shown in this image.
4. RESULTS AND DISCUSSION
4.1 A. Strains in the Ge Epi Film
In our design, n-type Si epi layer was first grown on the Si substrate at 950°C via epitaxial chemical vapor deposition. The heteroepitaxy of Ge on Si was initialized by a low-temperature deposited Ge seed layer at 400°C to minimize the dislocation densities at the Ge/Si interfaces [22,23]. Then, the growth temperature was raised to 700°C to grow the intrinsic and p-type Ge epi layers. Because of the different CTEs between Ge and Si substrate, a tensile strain can be formed in the Ge film upon cooling from the growth temperature to room temperature [20,35,36]. The strain states in our Ge layers were characterized by high-resolution XRD (HRXRD). Figure

Fig. 5. HRXRD (004) scan of Ge epi layers on Si substrate. The dashed line represents the peak of bulk Ge as a reference.
4.2 B. Improved EQE of Ge PDs by Photon-Trapping Holes
EQE, defined as the ratio of the number of charge carriers generated by incoming light to the number of incident photons, is an important metric to determine how sensitive a PD is. Measurements of EQE in Ge on Si PDs are performed using an NKT supercontinuum laser and a tunable filter that allows transmission of individual wavelengths with 5 nm resolution. A single-mode optical fiber was used to deliver the light to the PDs, and a semiconductor parameter analyzer (Agilent 4155B) was used to apply the electrical potential and measure the output current of the PDs. Dark current measurement was also performed under a no light illumination condition using the same setup. The EQEs of Ge-on-Si PDs with funnel-shaped photon-trapping holes (diameter, 1150 nm; period, 1750 nm; hexagonal lattice) were measured between the wavelengths of 1200 and 1800 nm. In addition, PDs without holes were also characterized for comparison. Figure

Fig. 6. (a) Measured EQEs of Ge-on-Si PDs with various designs of hole arrays at 1550 nm wavelength. The PD with hole arrays with diameter/period (d/p) of 1150/1750 nm in a hexagonal lattice has the best EQE. (b) Measured EQE versus calculated absorption of Ge-on-Si PDs with hole arrays (diameter/period: 1150/1750 nm) and without holes, for s wavelength range of 1200–1800 nm. Blue half-filled circles show measured EQE data of Ge-on-Si PDs with holes, while red half-filled circles represent measured EQEs of Ge-on-Si PD without holes. 73% EQE is recorded at 1550 nm wavelength with light-guiding holes, whereas the EQE of a PD without holes is only 47%. Red and black solid lines show the calculated absorption in PDs with and without holes, assuming 0.2% strained Ge on Si, respectively. Simulation results are in good agreement for PDs without holes, whereas PDs with holes are expected to show higher EQE at the longer wavelengths beyond 1600 nm. Such discrepancy can be attributed to the deviation in fabricated structures from design and recombination loss of photo-generated carriers. (c) Responsivity of Ge-on-Si PDs with and without holes for the wavelength range of 1200–1800 nm. 0.91 A/W responsivity is achieved at 1550 nm with holes. Inset: EQE enhancement by micro-/nanoholes, showing increase in EQE with holes for wavelengths beyond 1600 nm.
Figure
The enhancement in the EQEs of the Ge-on-Si PDs with photon-trapping holes is noticeable in both data communication wavelengths (1300 and 1550 nm). An 80% EQE is achieved at 1300 nm wavelength, whereas a PD without holes provides 65% EQE. A similar trend is observed at a wavelength of 1550 nm, where the EQE is improved from 43% to 73% by photon-trapping holes. Moreover, the enhancement in EQE is present over the entire broadband of wavelengths. As shown in Fig.
Another important figure of merit in PDs is responsivity, defined as the output electrical current of the PD produced in response to incident optical power. The inset in Fig.
4.3 C. Electrical Characterization
1. Dark Current Density
Since the photon-trapping holes are created by dry etching with physical bombardment of high-energy plasma ions, crystalline defects and dangling bonds can be formed at the Ge surface and create surface states that can contribute to increased dark current level, and thus need to be efficiently passivated. In this work, 10 nm

Fig. 7. (a) DCD of Ge PD devices of different mesa diameters without photon-trapping holes from to 1 V. (b) DCD of Ge PD devices of different mesa diameters with photon-trapping holes (1300 nm in diameter, and 2300 nm in period) from to 1 V (the dashed line shows the comparison with a 100 μm Ge PD without holes). (c) DCD of the PDs with different mesa sizes with different fill factors. (d) Schematic showing the definition of the fill factor in the PDs.
It should also be noted that the etched surface of a 100 μm device without holes is estimated to be around
2. High Speed
Ge-on-Si PDs were surface illuminated with a fiber probe that is connected to a fiber-coupled continuum laser filtered at 1300 nm. The optical pulse width was
Figure

Fig. 8. High-speed responses of a 30 μm PD with (blue) and without (black) holes, observed by a 20 GHz oscilloscope after illuminating the PD with a sub-picosecond optical pulse at 1300 nm wavelength. The insets show the simulated eye diagrams at the filter output for 10 Gb/s data transmission rate for PDs with and without holes.
A diffusion tail was observed after the pulse fall-time due to the slow carriers generated in the transition regions (p-i and i-n boundary regions). The dopant diffusion during epitaxial growth made the transition regions longer and softer where the applied field is not strong enough to drift the photo-generated carriers. This diffusion tail also verifies our hypothesis on the reduction of the effective i-layer in the FDTD simulation in Section
Another advantage of holes is to reduce the junction capacitance by decreasing the junction area [44]. That helps to reduce RC time, which is one of the factors limiting the speed of a pin diode. The capacitance reduction in our Ge-on-Si PDs with holes results in faster response compared to its counterpart without holes.
A computer simulation is used to evaluate the performance of Ge PDs with and without hole arrays for transmission speed. A pattern consisting of 2000 random bits was convolved with the PD impulse responses. The effect of a trans-impedance amplifier (TIA) was included in the simulations by applying the PD output to a third-order Butterworth filter with a 3 dB bandwidth equal to
5. CONCLUSIONS
A surface-illuminated Ge-on-Si photodiode with photon-trapping holes has been demonstrated in this work. The photon-trapping holes have enabled lateral propagation modes in the thin Ge layers and, thus, successfully improved the external quantum efficiencies of the PDs at wavelengths between 1200 and 1800 nm. EQEs of 80% and 73% (responsivity of 0.91 A/W) have been demonstrated at two data communication wavelengths (1300 and 1550 nm), respectively, both showing enhancement compared to Ge-on-Si PDs without holes. In addition, more than 3.5-fold increase in EQEs has been achieved at longer wavelengths beyond the L band (1620 nm), which makes these PDs attractive for a new data transmission window. The photon-trapping holes also reduce the temporal response of the PDs by reducing the junction capacitance. The Ge-on-Si PD with photon-trapping holes has an FWHM temporal response of 70 ps, which is suitable for 10 Gb/s data transmission operation. Our Ge-on-Si PDs with 2D arrays of high-density photon-trapping holes have the potential to be monolithically integrated with CMOS/BiCMOS ASICs. Such monolithic integration offers low-cost packaging solutions and allows low parasitics, resulting in high performance. Ge-on-Si PDs with photon-trapping holes can have applications for short- and long-reach communication at intra- and inter-datacenters, passive optical networks [3], lidar [47,48], and quantum communication systems [49], as well as enhancing the capacity of long-haul DWDM systems beyond the L band. Our future work will focus on optimization of the dopant diffusion between the highly doped and intrinsic regions of pin PDs to avoid a diffusion tail in the temporal response.
6 Acknowledgment
Acknowledgment. The authors thank S. P. Wang and S. Y. Wang partnership for the financial support.
Article Outline
Hilal Cansizoglu, Cesar Bartolo-Perez, Yang Gao, Ekaterina Ponizovskaya Devine, Soroush Ghandiparsi, Kazim G. Polat, Hasina H. Mamtaz, Toshishige Yamada, Aly F. Elrefaie, Shih-Yuan Wang, M. Saif Islam. Surface-illuminated photon-trapping high-speed Ge-on-Si photodiodes with improved efficiency up to 1700 nm[J]. Photonics Research, 2018, 6(7): 07000734.