Polariton lasing in InGaN quantum wells at room temperature
Exciton polaritons, bosonic quasi particles by strong coupling between photons and excitons, were firstly depicted by J. J. Hopfiled in 1950s1. The unique physical properties of light effective mass, tunable energy dispersions and convenient detection guaranteed their extensive research in nonlinear optics, low threshold lasing, quantum vortex and superfluid2.
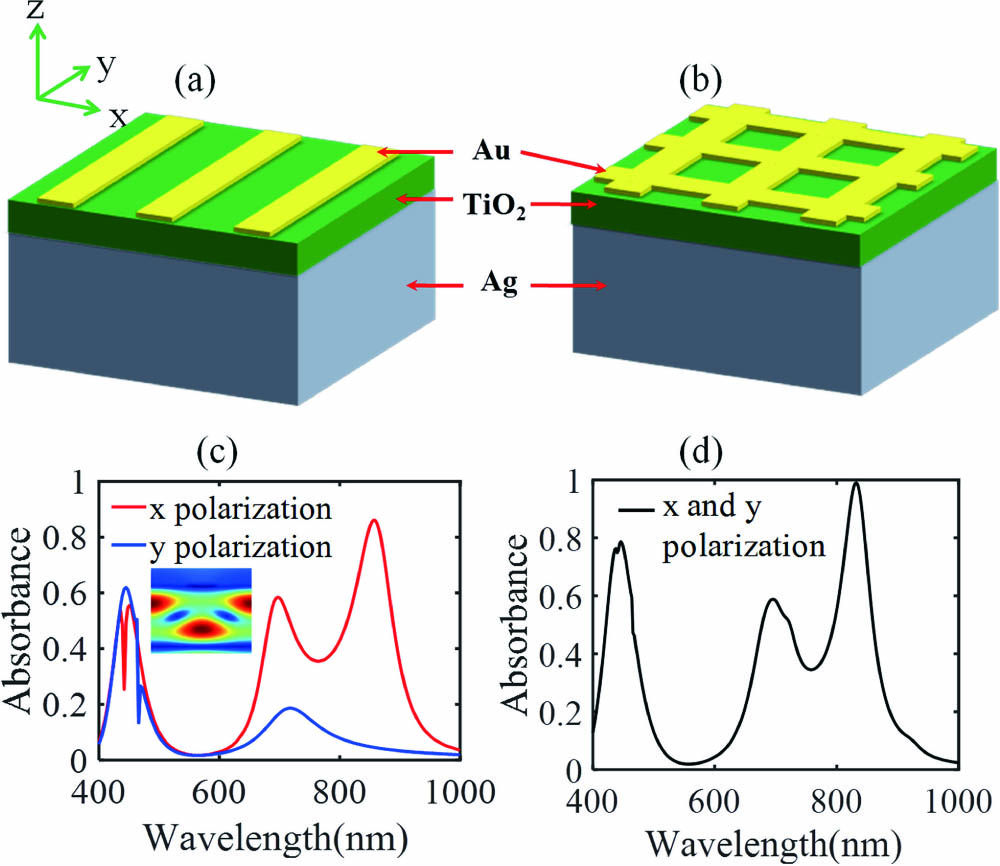
Fig. 2. (a ) Schematic of angle-resolved micro-PL setup. (b ) Schematic of angle-resolved PL Fourier image setup. (c ) The angle resolved micro-PL of positive detuning sample. (d ) LPB and UPB dispersion curves fitted by coupled oscillator model. (e ) The angle resolved micro-PL Fourier image of negative detuning sample.
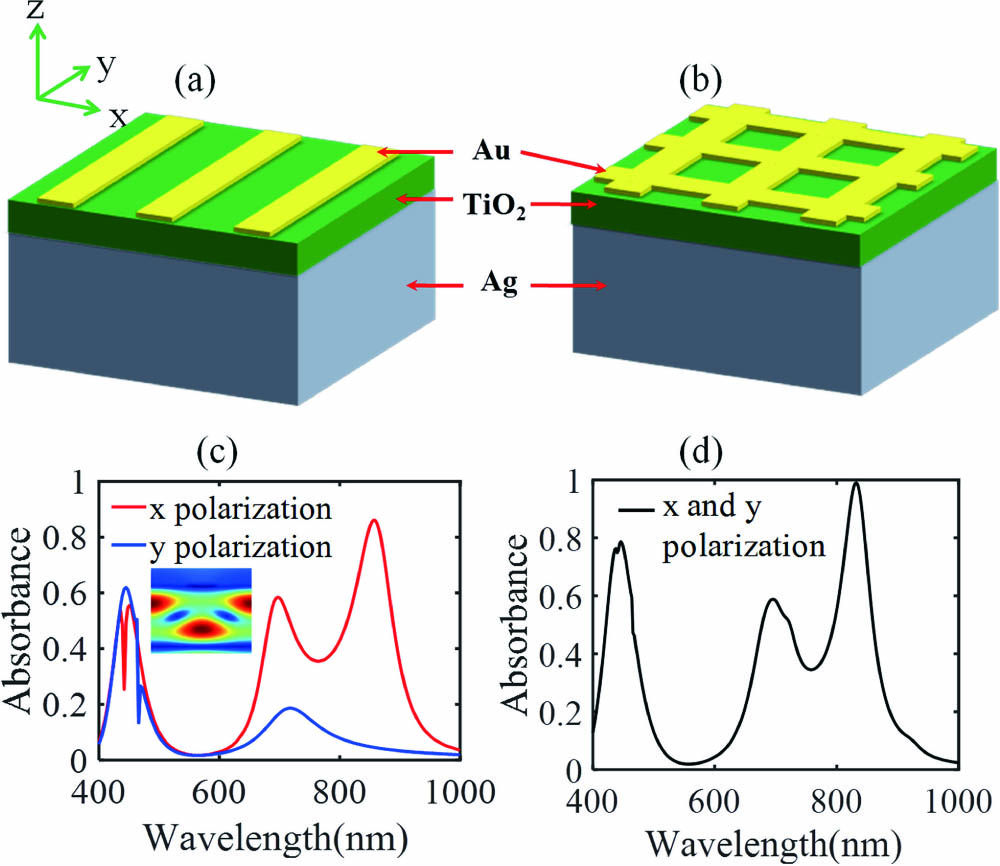
Fig. 1. (a ) Sample structure of InGaN/GaN MQWs microcavity for strong coupling. (b ) The optical field in the microcavity with MQWs placed on the antinode. (c ) and (d ) Photoluminescence spectrums of bare wafer at 15 K and 300 K.
Here, the studied microcavity structure (
However, in the positive detuning cavity, the lower polariton band (LPB) has large portion of exciton, which increases the mass of LPB and impedes polariton's condensation. The polariton lasing could not be observed by increasing excitation power in this sample.
In contrast with conventional photonic lasing, polariton lasing results from polaritons' condensation and is promising for the super-low threshold lasing due to its coherent spontaneous emission character3-5. The polariton lasing at room temperature (RT) based on GaN6, ZnO7, and perovskite8 have been demonstrated recently. Among them, GaN-based III nitride material systems are essential toward practical opto-electrical devices (LD, LED, etc.)9-11, with the ultraviolet to infrared full spectral coverage of Ga(In, Al)N alloys. In 2007, J. J. Baumberg et al. reported the first optically pumping polariton lasing in bulk GaN material6, followed by electrically injected polariton lasing in bulk GaN12 by P. Bhattacharya at RT in 2014. In 2004, T. Tawara et al. observed the exciton polariton in InGaN quantum wells (QWs) at room temperature by reflectance spectrum13. In 2011, T. C. Lu et al. successfully achieved the current injected InGaN MQWs polariton LED14. However, no polariton lasing in InGaN quantum wells has been reported yet, although the realization of InGaN based polariton lasing will be significant due to the vast application of InGaN in the solid-state lighting and lasing. Strong coupling in InGaN QWs system has been shown difficult since the large inhomogeneous broadening (due to Indium phase separation and well width fluctuation) and piezoelectrical field. In 2006 and 2014, N. Grandjean et al. theoretically anticipated that less than 46 meV inhomogeneous broadening was the prerequisite for the observation of exciton polariton lasing in InGaN QWs systems15, 16. They predicted that polariton lasing may be possible by combination of low Indium content and double-sides dielectric distributed Bragg reflectors (DBRs). Previously, we achieved giant Rabi splitting energy of 130 meV by coupled QWs and superior F-P cavity (Q > 3500)17.
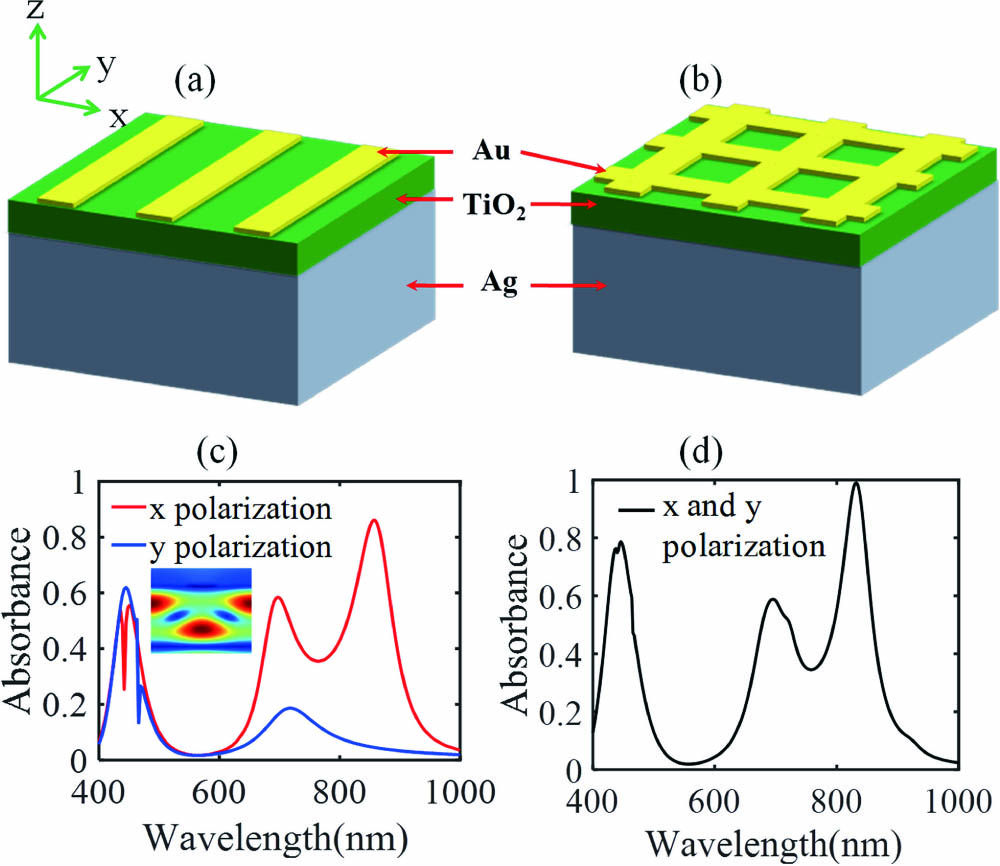
Fig. 4. The k-space mapping of exciton polaritons under different excitation power. (a) and (b) polariton (T1) and photonic (T2) lasing thresholds respectively.
To prospect the polariton distribution in k-space, the normalized angle-resolved PL mapping below and above the dual thresholds were recorded in
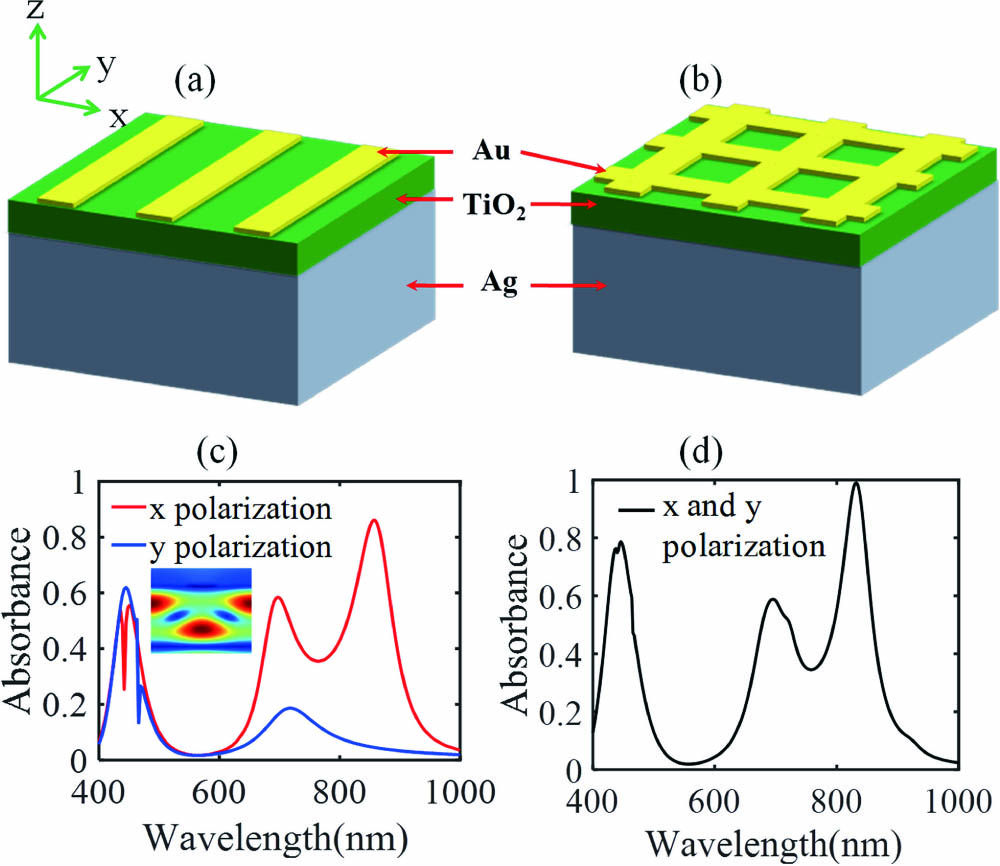
Fig. 3. (a ) The dependence of luminous intensity, peak position and half-width of LPB on the excitation power with double nonlinear regimes. (b ) Enlarged polariton lasing region with linear coordinates. (T1 and T2 denote the polariton lasing threshold and photonic lasing threshold)
For realizing polariton condensation, a negative detuning cavity was fabricated.
In positive detuning cavity (where the energy of cavity mode is larger than the exciton energy), two distinctive PL peaks were observed under 16 nJ/pulse excitation fluence as shown in
It is worth noting that exciton polariton lasing based on InGaN MQWs has not been reported before, due to the detrimental inhomogeneous broadening and piezoelectrical fields28. T. Tawara13 and T. C. Lu14 observed emission of the exciton polariton in InGaN MQWs by reflectance and electrical injection in 2004 and 2011, respectively. Although the polariton lasing in InGaN QWs was observed here in our study, the polariton lasing threshold was about half of the photonic lasing threshold. In previous reports on different materials with small exciton broadening, the polariton lasing thresholds are one or two magnitudes smaller than the photonic lasing threshold. Therefore, the polariton lasing threshold observed in our study was still higher than expected. This large polariton lasing threshold may originate from the large negative detuning value and severe exciton broadening in InGaN MQWs. As reported in GaN/AlGaN MQWs structures, an optimum negative detuning value for the lowest polariton lasing threshold results from the trade-off between thermodynamics and relaxation kinetics29, 30. LPB with large negative detuning yields large portion of photons, which inhibits the LPB condensation and increases the polariton lasing threshold due to the reduced polariton scattering. Meanwhile, the half-width broadening of InGaN MQWs is also essential in polariton lasing action. Only those excitons whose energies match the cavity photon energy are picked out and contribute to the strong coupling and polariton lasing. Therefore, the large inhomogeneous broadening of 43 meV in our sample should also significantly increase the polariton lasing threshold.
In this work, we reported the first room temperature polariton lasing in InGaN/GaN multi-quantum wells (MQWs) by further thinning the cavity length to 4.5λ F-P cavity structure sandwiched by two dielectric DBRs. By adjusting the cavity length, strong coupling effects were observed in positive and negative detuning scenarios respectively. Dual thresholds corresponding to polariton lasing and photonic lasing were clearly observed in negative detuning cavity. The nonlinear optical properties, half-width narrowing and peak blue-shifts were also confirmed. The gap between these two lasing thresholds was smaller compared with GaAs and GaN based binary crystal systems, which should be contributed to the severe inhomogeneous broadening and large negative detuning value.
In summary, strong coupling and polariton lasing was observed in InGaN MQWs embedded in 4.5λ microcavity at room temperature. Dual thresholds corresponding respectively to the polariton lasing and photonic lasing were clearly observed. The polariton lasing threshold was half of the photonic lasing threshold. Large negative detuning value and severe inhomogeneous broadening of exciton in InGaN MQWs are considered to be important factors in determining the lasing threshold of polariton. Nevertheless, we believe that our work will pave a way for ultra-low threshold InGaN QWs lasers and Boson-Einstein Condensate (BEC).
2 Acknowledgements
This work was supported by the National Key Research and Development Program of China (No. 2016YFB0400803), the Science Challenge Project (No. TZ2016003), and the National Natural Science Foundation of China (Nos. 61704140, U1505253).
3 Competing interests
The authors declare no competing financial interests.
Article Outline
Jinzhao Wu, Hao Long, Xiaoling Shi, Song Luo, Zhanghai Chen, Zhechuan Feng, Leiying Ying, Zhiwei Zheng, Baoping Zhang. Polariton lasing in InGaN quantum wells at room temperature[J]. Opto-Electronic Advances, 2019, 2(12): 12190014.